Gengzhi Goblets Overview
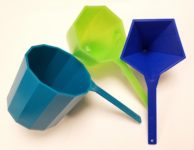
Welcome to Studio Infinity! You most likely reached this page via the link on the Gengzhi Goblets given away at G4G13. Here are pointers to more in-depth discussions of the Goblets.
Read MoreWelcome to Studio Infinity! You most likely reached this page via the link on the Gengzhi Goblets given away at G4G13. Here are pointers to more in-depth discussions of the Goblets.
Read MoreSo far, we’ve created a lot of interesting small models of tensegrity structures. However, for doing public programs of the sort Storm King Art Center was planning, it’s always helpful to be able to build much larger models of things. Building giant models seems to get the ideas across more vividly, engage visitors more thoroughly, and be just plain fun.
Read MoreSo why the interest in tensegrity here at Studio Infinity? It begins with Kenneth Snelson, the inventor of tensegrity (although perhaps not of the term) as a student of Buckminster Fuller. Ken went on to become a noted sculptor, using tensegrity in many of his works. One of those sculptures, Free Ride Home, resides at Storm King Art Center. And recently, the education department at Storm King Art Center invited me to give a workshop there about tensegrity, how to make simple models of tensegrity, and how to share ideas about tensegrity with the public visiting the Center.
Read MoreWhen I set the goal of creating a new tensegrity structure for the Storm King Art Center workshop, I decided that I wanted to create a highly regular, symmetric structure, to contrast with the more free-form, organic style of Snelson’s Free Ride Home at the Center. (Snelson also created many very regular tensegrity sculptures himself.)
As is often the case, the creative process began with an existing structure that shared many of the characteristics I wanted, namely the classic six-strut tensegrity structure. In a previous article, we saw how that structure is based on a regular icosahedron; in fact, the compression members are just six diagonals of an icosahedron that are equivalent under the symmetries of the icosahedron. Moreover, the end points of the diagonals exhaust the twelve vertices of the icosahedron.
Thus, it seemed that I might obtain a different interesting structure if I started with a different polyhedron that shared some key properties with the icosahedron, and then looked for an appropriate set of diagonals. So the first question was, what are those key properties of the icosahedron? Well, we want all of the edges of the shape to be the “same” in some sense so that when we fabricate them from tension members with the same tension, they will balance each other uniformly. And what does “same” mean in this context? Well, a natural answer is that there should be some symmetry of the shape — in other words, some shape-preserving transformation, which in this case will be a rotation — which will match any edge up with any other edge. When the symmetries of an object completely scramble some component of that object, mathematicians call the object “transitive” for that component. So I could find a list of candidate shapes by an internet search for “edge-transitive polyhedron.” That search turned up a fancy synonym for edge-transitive, namely “isotoxal,” and it also turned up this Wikipedia page, of which the first table is of particular interest.
The rhombic triacontahedron immediately caught my eye. However, it includes two different categories of vertices, the ones where five edges meet and the ones where three edges meet; and what’s worse, there are twelve of the former but twenty of the latter. That means there will be no way to make sixteen equivalent diagonals, since some of them will not terminate at a five-edge vertex at either end, while others will.
So instead, I set my sights on it’s left-hand neighbor in that table, the icosidodecahedron. It has 30 identical vertices (consistent with our theme, the symmetries of the icosidodecahedron act transitively on the vertices). So then I searched for a set of fifteen diagonals of the icosidodecahedron, all the same length, to be the struts of the new tensegrity.
I began with what seemed like the simplest sort of diagonal, namely a diagonal between two vertices that are only “two edges apart,” i.e., both adjacent to the same vertex. I was able to draw a set of diagonals that exhausted the vertices, like this:
However, there’s a problem: you can see on the right-hand side of the image that two of the diagonals selected intersect. While that’s OK mathematically, there’s no way to direct struts to pass through each other. And try as I might, I couldn’t avoid two of these diagonals intersecting. From a math point of view, the natural response was to try to prove that any such set of diagonals contains (at least) two that intersect each other. That turns out to be the case, and here’s how the proof goes: Any diagonal that connects two vertices that are adjacent to the same vertex is actually a diagonal of one of the pentagonal faces of the icosidodecahedron. There are only twelve such faces, but we need 15 diagonals. Hence, at least one of the faces will end up with two diagonals. And (as you can demonstrate for yourself by drawing some diagrams) any two diagonals of a regular pentagon intersect.
So these “simplest” diagonals were out. And it also occurred to me that for building a tensegrity structure, we likely want long diagonals that go through the body of the polyhedron, to make it easier for the tension of the rubber bands along the edges of the polyhedron to pull against them. The very longest diagonals of the icosidodecahedron all intersect each other at the center of the polyhedron, so they’re out. Therefore, my next attempt was to use the second-longest diagonals, like this partial effort:
You can see that this selection of diagonals already has intersecting pairs. In fact, I was not able to find any selection of diagonals that fit the requirements above, leading to this Math StackExchange question. So what did I do? I settled for the “near miss” below. The ten diagonals that connect a “polar” vertex to an “equatorial” vertex are transitive, but there’s no way to transform them to one of the other five diagonals (that connect two “mid-latitude” vertices, one above the equator and one below.) In fact, the two types of diagonals are different lengths, with the first type about 20
So this image became the template for building the new tensegrity structure, which you can read about in the next MakeStream post.
OK, so now we are going to try to force a tensegrity structure to take on the shape of a truly regular icosahedron. But we don’t have to search blindly for a way; we are armed with the results of this MathStream post about how to do it.
Read MoreAs mentioned at the end of the last MathStream post, the actual shape that the six-strut tensegrity structure takes on is close to, but not quite precisely, a regular icosahedron. And that fact immediately makes you want to build a tensegrity structure that will under ideal circumstances assume the shape of a truly regular icosahedron. What would that entail? Why is the classic tensegrity not a regular icosahedron? One possibility that immediately comes to mind is that not all of the edges of the icosahedron are represented in the same physical way in the model. Namely, as you may recall from this diagram,
there is no rubber band lying along some of the edges of the icosahedron. To test if that’s the reason that the six-strut is not regular, we’d want a different arrangement of elements so that there will be exactly the same tension on a rubber-band connection between every closest pair of endpoints of the six struts, as shown in this diagram.
So, you might immediately start tying to build such a configuration. But that will bounce you right back to a mathematical question: how can we route the rubber bands so that there is exactly the same amount of rubber band between every closest-neighbor pair of endpoints? In fact, one of the wonderful things that happens at Studio Infinity is that many of the things we try to build lead to new mathematical questions, and many of the mathematical discoveries we encounter suggest new things to build. So the MakeStream and MathStream really build on each other.
Getting back to the question at hand, it amounts to finding a route for each of several rubber bands so that the route of each band traverses the same number of edges of the icosahedron we are trying to achieve, and so that every edge is covered. Since the icosahedron has thirty edges, that immediately narrows down the possible number of rubber bands we might use. For example, there might be ten rubber bands each covering three edges, or six rubber bands each covering five edges, or five rubber bands each covering six edges, and so on. And since each rubber band is a loop, the edges covered will have to form a loop on the surface of the icosahedron. For example, there are fairly obvious loops one could make with three edges (in blue) or with five edges (in red) in this diagram.
So you can just start trying to cover the edges with loops of the same length. In fact, I recommend that you give it a try right now, maybe using the six-strut model you’ve made, or the diagram above. See if you can find a way to route rubber bands to cover every edge, so that every loop is the same length, before you read further.
After a while of trying, you may start to get discouraged. You might start to feel that this is an impossible task. And that would not be too surprising, because it is impossible. But when we make a statement like that in mathematics, we have to back it up. If you want to claim that something is impossible, you can’t just list the seventeen things you tried that didn’t work. You’ve got to find reasons why no possible routing of rubber bands, no matter how clever, will cover all of the edges and have every loop be the same length.
And the key to that in this case is even and odd. First count how many edges meet at every vertex: five, an odd number. Next count how many of those edges rubber bands can cover. Well, every rubber band is a loop, so it must arrive at the vertex by some edge, and then leave that vertex by a different edge. It might later come back to that vertex by yet another edge, but if so, it has to leave again by a still further edge, in order to form a single closed loop overall. So in short any one rubber band covers an even number of edges touching the vertex. And since the sum of a collection of even numbers is even, we can be sure that the rubber bands are covering an even number of edges touching the vertex.
And so now we run into the problem. An even number cannot be equal to an odd number, so we can’t possibly have covered all of the edges. So there in fact is no way to route the rubber bands as desired.
So we regretfully conclude that there is no way to construct the regular icosahedron we are looking for, right? Not quite. Another habit in mathematics is, when something doesn’t work out the way you thought it might, to see if there’s a way to change the assumptions so that it does work out. And in this case, there is something we can change: rather than providing the tension between two neighboring endpoints by virtue of a single segment of rubber band, why couldn’t there be exactly two different (but identical in length) segments of rubber band covering each edge? Then there would be ten different segments of rubber band reaching each vertex, which is an even number, and the contradiction underlying our impossibility argument would melt away in a puff of logic.
And now we can very pleasantly notice that there are exactly twelve of the five-edge pentagonal paths similar to the one highlighted in red in the last diagram above, and that each edge lies on exactly two of these pentagonal paths. So now the plan is simple: connect one rubber band along each pentagon, and the icosahedron should simply materialize from the balance of forces. Let’s return to the MakeStream to see if it works…
So maybe you’ve made the classic six-strut tensegrity (or perhaps you’ve just looked at the pictures) and you’re wondering what shape that is, exactly. Naturally, since mathematics is among other things the science of shape and structure, understanding that is going to involve a little math. And in mathematics, sometimes it’s easiest to understand something by breaking it down into the pieces that are there, but other times it’s actually easier to understand something by adding (or pretending to add) pieces that aren’t there.
So take a look again at the structure you built.
Notice that in many cases, two neighboring endpoints of struts are connected by segments of rubber band. However, in other cases, there are endpoints of two struts that are close together but are not connected at all. So let’s imagine what the structure would look like if all of the nearest neighbors were connected.
Now, if we look at the outer surface of the structure, we see a much simpler pattern. Every outermost flat shape of the structure — which we call a face of the structure — is a triangle. There are 20 of these triangles, and at each endpoint of a strut, which makes a sort of “corner” of the structure called a vertex, exactly five of the triangles meet. There’s just one geometric shape fitting those facts, called the icosahedron.
This shape is simple and natural enough to have been discovered at least two and a half millennia ago, as one of the five Platonic Solids of the ancient Greeks. These are the shapes with identical regular polygonal faces and identical vertices, and it is a remarkable and beautiful fact that only five different Platonic solids exist. Yet the icosahedron is also intriguing and pleasing enough that it’s still of interest today.
One of the most important characteristics of the icosahedron is that it has very many symmetries. A symmetry of an object is some transformation, like a rotation or a translation or some combination thereof, which brings the shape back to coincide with itself. For example, if we were to rotate the icosahedron one-fifth of a turn around the axis indicated by the dotted blue line in this diagram, it would again look exactly the same, even though we moved it.
In the idealized world of this Platonic icosahedron, how can we represent the struts? Each one of them connects two of the vertices of the icosahedron, so it is what you might call a diagonal of the icosahedron. Let’s draw all six of them on our diagram.
Note how these diagonals are arranged; each one connects a vertex to another vertex that’s not adjacent, but lies “two edges away” from the starting vertex. They come in three pairs of parallel diagonals. Moreover, all six diagonals are “equivalent” under the symmetries of the icosahedron, which means to say that there’s a symmetry of the icosahedron which moves any of the struts to the position of any one of the other struts. That corresponds with the fact that all of our struts are the same length: in order to get an icosahedron, it’s necessary to use struts that are the same length, because there’s no way to tell one strut from another. They’re all interchangeable by the symmetries of the icosahedron.
We’ll close this tour of the math underlying the six-strut tensegrity with a perhaps inconvenient-seeming observation. If you look closely at the structure you’ve built (or at the pictures above), you will see that the icosahedron is not actually perfectly regular. In the diagram, the dotted sides of the triangles are not the same lengths as the rubber-band sides of the triangles. So our tensegrity structure is not actually a Platonic regular icosahedron; you might instead dub it an “icloseahedron” since it’s really close to, but not quite identical to, the idealized structure we envisioned.
But why isn’t this structure regular? After all, we built it in a very symmetric way. Well, there’s one way in which the physical structure of the six-strut tensegrity differs markedly from a true icosahedron — and we’ll take that up in the next MathStream article.
HERE!! go on about symmetries, show how the struts relate, point out that the tensegrity isn’t precisely a regular icosahedron, end with wondering why, saying that will have to wait for another post.
Studio Infinity is kicking off with a group of articles that feature the concept of tensegrity. What does that mean?
Read MoreHere’s a closeup of some snub dodecahedra that students built as part of a group construction I led at The College of New Jersey in July of 2017. They are each approximately 80cm in diameter.
Read MoreHere are two large-scale group constructions I facilitated the construction of as part of a session at a 2017 MAA Spring Sectional meeting at Frostburg University. The first is the one I have dubbed the “Octahex Ring” — it’s a compound of a dozen octahedra:
Read More